A page from the "Causes of Color" exhibit...
What causes the colors of metals like gold?
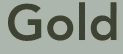
The lure of gold has been the downfall of many, from those worshipping the biblical golden calf to those unsuccessfully staking their claims during the 19th century gold rushes. Nevertheless, this lustrous metal continues to connote the pinnacle of achievement, as evidenced by Nobel Prize medals, Olympic medals, and Academy Award statuettes. Would our fascination with gold be lessened if we knew that its shiny allure was the result of excited electrons?
Silver, iron, platinum, gold, and copper are all metals, which generally are malleable and ductile, conduct electricity and heat, and have a metallic luster. Some of their properties can be attributed to the way electrons are arranged in the material.
![]() This piece of jewelry is framed in yellow gold, with purple gold detail on the right border. |
![]() This illustrated manuscript from the Book of Hours is decorated with gold leaf, beaten to an extremely thin foil. |
In this hypothesis, each metal atom contributes its outer electrons to a shared pool. The electrons are free to move throughout the metal, which explains its high electrical and thermal conductivities and luster.
The bonding of metals
When two atoms combine, different types of bonding can occur: covalent, ionic, and metallic. Silver, iron, platinum, gold, and copper all form metallic bonds. Unlike covalent bonding, metallic bonding is non-directional. The strong bond consists of positively charged metal atoms in fixed positions, surrounded by delocalized electrons. These delocalized electrons are often referred to as "a sea of electrons," and can help explain why copper and gold are yellow and orange, while most other metals are silver.
When light strikes the surface of a metal, electrons in a lower energy level can be excited to a higher energy level. The distance between the levels represents the relative energy required to excite an electron. When four atoms combine, the outermost energy levels merge, providing four energy levels at a low energy and four energy levels at a higher energy. As the number of neighboring atoms increases, the spacing between the energy levels decreases. More overlap occurs and bands of low and high energy replace the distinct energy levels. As more atoms combine, the distance between the two bands decreases, the band gap decreases, and less energy is required for the electron to be excited from one band to the other. In metals, when very large numbers of atoms are brought close to each other, the low and high energy bands can overlap, forming a nearly continuous band of available energy levels, where electrons may move freely.
Band Theory
The color of metals can be explained by band theory, which assumes that overlapping energy levels form bands.
The mobility of electrons exposed to an electric field depends on the width of the energy bands, and their proximity to other electrons. In metallic substances, empty bands can overlap with bands containing electrons. The electrons of a particular atom are able to move to what would normally be a higher-level state, with little or no additional energy. The outer electrons are said to be "free," and ready to move in the presence of an electric field.
Some substances do not experience band overlap, no matter how many atoms are in close proximity. For these substances, a large gap remains between the highest band containing electrons (the valence band) and the next band, which is empty (the conduction band). As a result, valence electrons are bound to a particular atom and cannot become mobile without a significant amount of energy being made available. These substances are electrical insulators. Semiconductors are similar, except that the gap is smaller, falling between these two extremes.
The highest energy level occupied by electrons is called the Fermi energy, Fermi level, or Fermi surface.
Above the Fermi level, energy levels are empty (empty at absolute zero), and can accept excited electrons. The surface of a metal can absorb all wavelengths of incident light, and excited electrons jump to a higher unoccupied energy level. This creates current, which rapidly discharges to emit a photon of light of the same wavelength. So, most of the incident light is immediately re-emitted at the surface, creating the metallic luster we see in gold, silver, copper, and other metals. This is why most metals are white or silver, and a smooth surface will be highly reflective, since it does not allow light to penetrate deeply.
If the efficiency of absorption and re-emission is approximately equal at all optical energies, then all the different colors in white light will be reflected equally well. This leads to the silver color of polished iron and silver surfaces.
The efficiency of this emission process depends on selection rules. However, even when the energy supplied is sufficient, and an energy level transition is permitted by the selection rules, this transition may not yield appreciable absorption. This can happen because the energy level accommodates a small number of electrons.
For most metals, a single continuous band extends through to high energies. Inside this band, each energy level accommodates only so many electrons (we call this the density of states). The available electrons fill the band structure to the level of the Fermi surface and the density of states varies as energy increases (the shape is based on which energy levels broaden to form the various parts of the band).
If the efficiency decreases with increasing energy, as is the case for gold and copper, the reduced reflectivity at the blue end of the spectrum produces yellow and reddish colors.
Metals are colored because the absorption and re-emission of light are dependent on wavelength. Gold and copper have low reflectivity at short wavelengths, and yellow and red are preferentially reflected, as the color here suggests. Silver has good reflectivity that does not vary with wavelength, and therefore appears very close to white.
Silver, gold and copper have similar electron configurations, but we perceive them as having quite distinct colors. Electrons absorb energy from incident light, and are excited from lower energy levels to higher, vacant energy levels. The excited electrons can then return to the lower energies and emit the difference of energy as a photon.
If an energy level (like the 3d band) holds many more electrons (than other energy levels) then the excitation of electrons from this highly occupied level to above the Fermi level will become quite important. Gold fulfills all the requirements for an intense absorption of light with energy of 2.3 eV (from the 3d band to above the Fermi level). The color we see is yellow, as the corresponding wavelengths are re-emitted. Copper has a strong absorption at a slightly lower energy, with orange being most strongly absorbed and re-emitted. In silver, the absorption peak lies in the ultraviolet region, at about 4 eV. As a result, silver maintains high reflectivity evenly across the visible spectrum, and we see it as a pure white. The lower energies (which in this case contain energies corresponding to the entire visible spectrum of color) are equally absorbed and re-emitted.
Silver and aluminum powders appear black because the white light that has been re-emitted is absorbed by nearby grains of powder and no light reaches the eye.
Showing the variation of density of states and the excitation seen in gold, silver and copper (actually from the 3d band to above the Fermi level) |
The blue end of the spectrum is at a higher energy level than yellow and orange. |
Transmitted color of gold
Gold is so malleable that it can be beaten into gold leaf less than 100 nm thick, revealing a bluish-green color when light is transmitted through it. Gold reflects yellow and red, but not blue or blue-green. The direct transmission of light through a metal in the absence of reflection is observed only in rare instances.
Colored gold alloys
When two metals are dissolved in each other (as is the case with alloys), the color is often a mixture of the two. For example, copper dissolved in gold changes the color from a yellow-gold to a red-gold. Silver dissolved in gold creates a green-gold color. White gold contains palladium and silver. The color of gold jewelry can be attributed to the addition of different amounts of several metals (such as copper, silver, zinc, and so on). Some of these color changes can be explained by shifts in the energy levels relative to the Fermi level.
![]() This platinum ring has rose gold flowers with diamond centers to either side of the central diamond, and leaves of green gold. |
![]() These 18 karat gold bands contrast the hues of white, pink and green gold. |
Some alloys form intermetallics, where strong covalent bonds replace metallic bonding. Bonding is localized, so there is no sea of electrons.
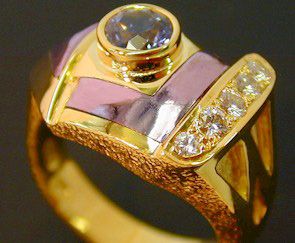
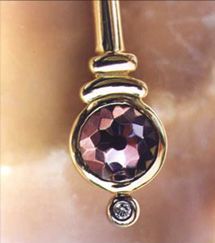
The addition of aluminum to gold creates a brittle purple gold. Purple gold used in a pin, and a purple gold inlay within a yellow gold ring.
When indium or gallium is added to gold, a blue color can result. The cause of color in these intermetallics is different than that of yellow gold.
Surface colors
Many metals create the illusion of being colored. The color can be attributed to a very thin surface coating, such as a paint or dye, or thin oxide layers can create interference colors (see butterflies) similar to those in oil or soap bubbles.
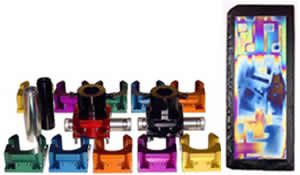
Some metals like aluminum (left) and titanium (right) can be anodized to create colors. The anodizing process creates a thin oxide layer. Aluminum and titanium films produced by anodizing can form thin, transparent coatings that produce interference effects in reflected light. The colors are applied using a bath, a brush, or a sponge, with the voltage applied determining the final color. Another anodizing application, particularly in aluminum, is the formation of thick, porous coatings that can absorb dyes to produce intense colors.
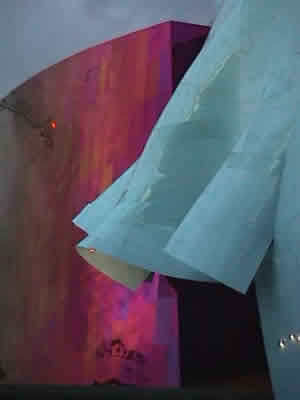
Stainless steel can also be colored, the color varying with oxide thickness, but the anodizing process is not used. In Sky Church, the purple area consists of stainless steel, coated to create interference effects. The panels appearing to flutter above the path of the monorail speeding below are made from painted aluminum.
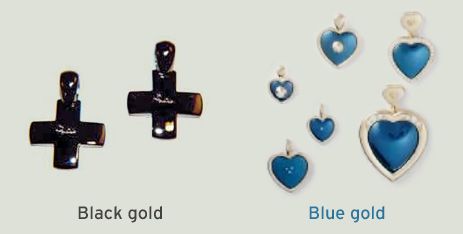
Gold can be colored by creating surface oxide layers. Because gold does not oxidize in its pure form, base metals have to be added to create blue, brown, and black gold. The "Hearts" collection, in blue gold, is by Ludwig Muller of Switzerland. Crosses of black gold may be colored in different ways, as shown above.
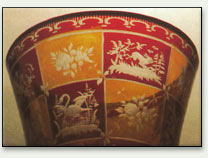
This antique engraved Czechoslovakian glass shows color produced by colloidal suspensions. When gold is in metallic colloidal form, as in the 10-nm-diameter particles in "ruby glass," the very complex "Mie scattering theory" has to be used to explain the unexpected red color illustrated; the yellow glass in this figure is colored by Mie scattering from metallic colloidal silver particles.
The color of nanoparticles
The color known as "Purple of Cassius" in glass and glass enamel is created by incorporating a colloidal suspension of gold nanoparticles, a technology in use since ancient times. Colloidal silver is yellow, and alloys of gold and silver create shades of purple-red and pink.
Nanoshells are a recent product from the field of nanotechnology. A dielectric core is coated with metal, and a plasmon resonance mechanism creates color, the wavelength depending on the ratio of coating thickness to core size. For gold, a purple color gives way to greens and blues as the coating shell is made thinner. In the future, jewelry applications may include other precious metals, such as platinum.
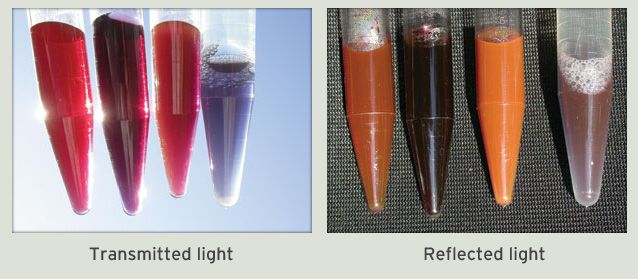
Dispersions of discrete gold nanoparticles in transparent media provide a fascinating range of colors, only recently exploited in the manufacture of paints and coatings. The shape of the particles and the viewing conditions determine the color we see. The gold particles in the test tubes on the left are shown in transmitted light, while the image on the right shows the same gold nanoparticles viewed in reflected light.
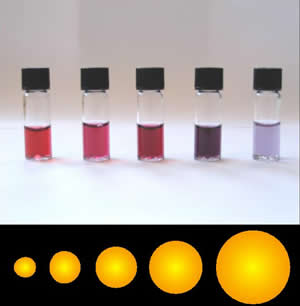
The diameter of gold nanoparticles determines the wavelengths of light absorbed. The colors in this diagram illustrate this effect.
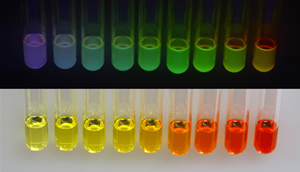
Different sized quantum dot nanoparticles are shown above, first in ultraviolet light and then in ambient light. The length of the synthesis reaction determines particle size for CdSe, increasing from left to right. In colloidal suspension, this semiconductor behaves in the same way as a metal.